Are midlatitude weather and climate influenced by Arctic Amplification?
The Arctic region has undergone a stronger surface warming in recent decades than most other regions of the world. This Arctic Amplification – the excess of Arctic warming with respect to the global mean warming – is associated with an unprecedented Arctic sea ice loss. It has been proposed that the Arctic Amplification, by decreasing the equator-to-pole temperature contrast, could lead to changes in the upper-tropospheric jet stream, planetary waves, and storm tracks. The profound changes in the Arctic also appear to be accompanied by an observed increase in extreme weather events in the mid-latitudes. Unusually cold winters in North America and Eurasia have been observed, while the winters in the Arctic remained relatively mild. Particularly the anomalous winter cooling over Eurasia has been associated to warming over the Barents-Kara Seas, a pattern of variability known as the Warm Arctic Cold Siberia (WACS) pattern (Fig. 1, left panel).
Whether the WACS is a forced pattern or a manifestation of natural variability has been disputed (Doblas-Reyes et al., 2021). Scientists at the Max Planck Institute for Meteorology (MPI-M) have explored with observational analyses the role of atmospheric blocking in mediating the links at various time scales between Arctic sea ice loss and more frequent cold surges in mid-latitudes. The weather in the temperate zones is characterized by changeable conditions driven by low- and high-pressure systems that are important for the maintenance of the energy balance of the planet. These systems mainly grow over the oceans and travel eastwards, giving rise to the well-known storm tracks. At times, the high-pressure systems become stationary, and a blocking pattern emerges. The eastward progression of weather systems is then obstructed; the flow becomes easterly and in addition acquires a strong meridional component. This weather disruption associated with atmospheric blocking can persist for periods longer than a week. As a result, the intense and prolonged equatorward advection of air masses of polar origin and the poleward advection of air masses of subtropical origin over long distances can instigate extreme winter cold spells and severe summer heatwaves over the mid-latitudes, respectively.
The analysis of the MPI-M scientists based on observational and reanalysis data has revealed a robust link between Ural Blocking (UB) and the WACS pattern on daily to sub-seasonal timescales (Fig. 2, upper panel, Tyrlis et al., 2020). Surprisingly, at these timescales, UB controls the pace of the WACS; warming over the Barents-Kara Seas and cooling over Central Asia peak 3–5 days after the UB onset (Fig. 2, left and middle panel). The Barents-Kara Sea as well warms and loses sea ice after the UB onset (Fig. 2, right panel). The observed sea ice deficit over the Barents-Kara Seas in the weeks prior to UB onset is not statistically significant when the long-term trend in sea ice is removed. The fast build-up and decay of the anomalous surface heat fluxes, which follow the evolution of the near surface temperature anomalies, is an indication that sea ice variability on short timescales is driven by the atmosphere (Tyrlis et al., 2020). Thus, on synoptic to sub-seasonal timescales, the sea ice deficit does not have a direct impact on UB occurrence but develops instead as a delayed response to UB. The interannual variability of WACS is also strongly linked to UB.
A characteristic example of Arctic sea ice loss that is driven by UB-induced atmospheric circulation refers to autumn and early winter 2016–2017, a period dominated by repeated cold surges over mid-latitude Eurasia, exceptionally warm conditions and sea ice loss over the Arctic, and the unseasonable weakening of the stratospheric polar vortex. Analysis of observational/reanalysis data has shed light on the dynamical pathways that caused these extreme phenomena. Following abnormally low sea ice conditions in early autumn over the Pacific sector of the Arctic basin, blocking anticyclones became dominant over Eurasia throughout autumn. UB activity was four times above climatological levels and organized in several successive events. UB episodes played a key role in the unprecedented sea ice loss observed in late autumn 2016 over the Barents-Kara Seas and the weakening of the stratospheric vortex (Tyrlis et al., 2019). Each block induced circulation anomalies that resulted in cold-air advection to its south and warm-air advection to its north. The near-surface warming anomalies over the Arctic and cooling anomalies over mid-latitude Eurasia varied in phase with the life cycles of UB episodes.
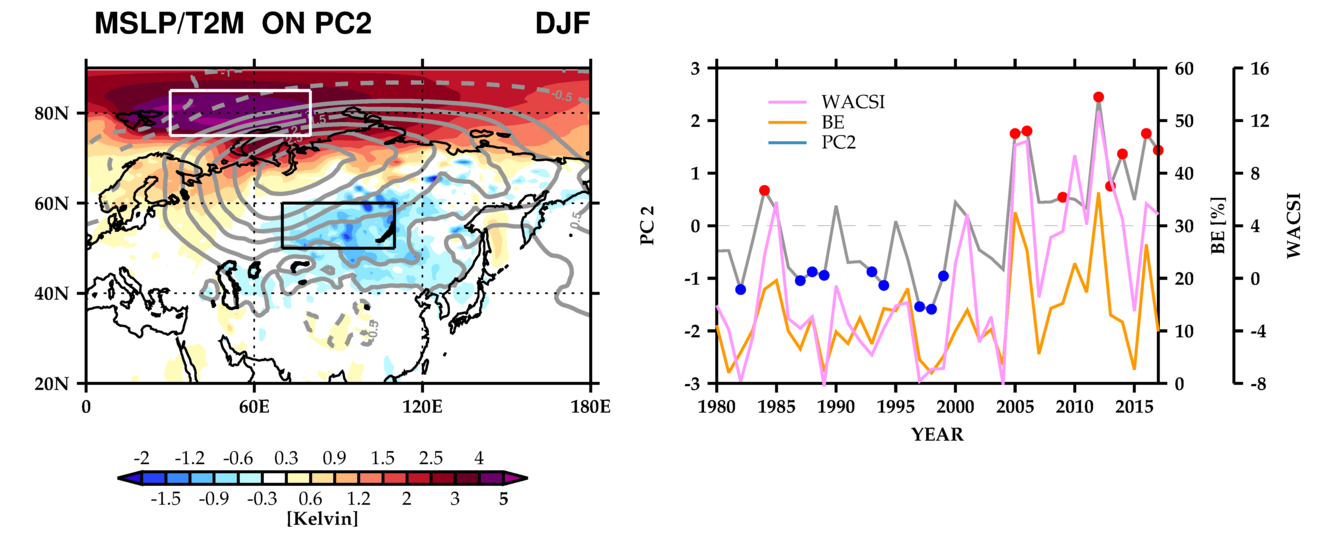
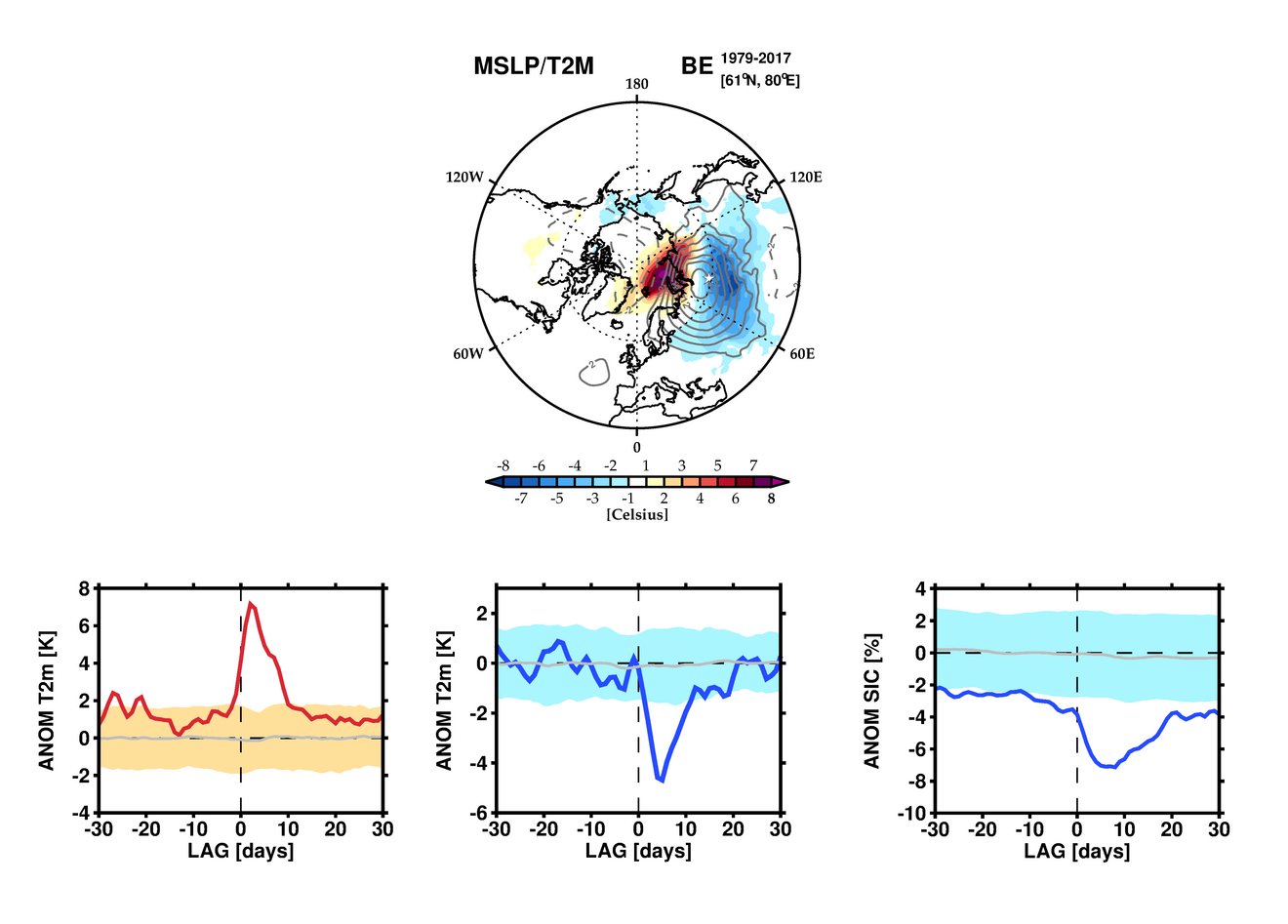
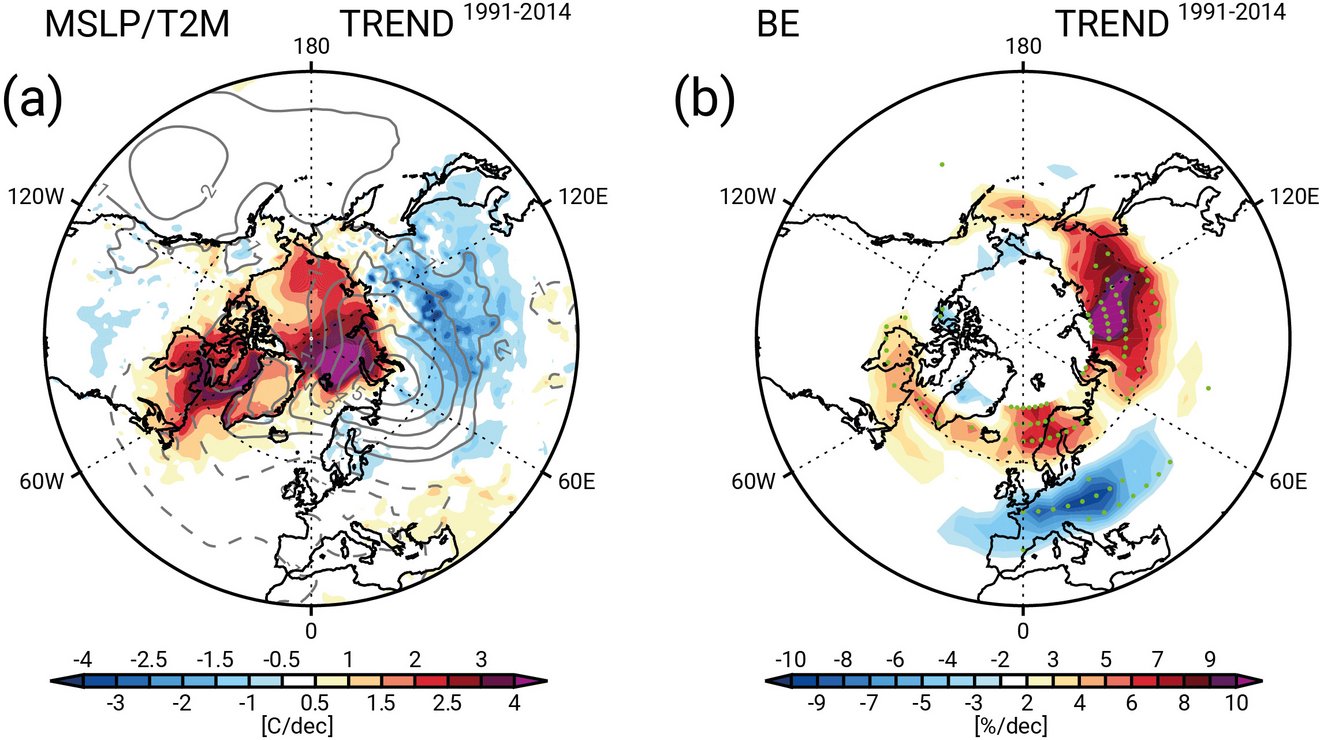
The sea ice cover minimum over the Barents-Kara Seas in 2016 was not observed in late summer but rather in mid-November and December, shortly after the two strongest UB episodes. Each UB episode drove an intense upward flux of wave activity that resulted in unseasonable weakening of the stratospheric vortex in November. The surface impact of this weakening can be linked to the migration of blocking activity and cold spells toward Europe in early winter 2017.
Particularly unclear is the role of the sea ice decline over the Barents-Kara Seas emerging after the late 1990s in changing the frequency and persistence of blocking and so leading to a Eurasian cooling via the WACS. In the observations, the MPI-M scientists have identified a significant upward trend in wintertime frequency of UB occurrence in recent decades that accounts for a cooling rate of 1oC/decade over Central Asia (Figs. 1; right panel, Fig. 3). Over the Barents-Kara Seas, UB trends explain a small fraction of the warming, which is dominated by Arctic Amplification (Fig. 3). Whether the long-timescale changes in the Arctic have driven the UB trend remains an open question; the observational studies suffer from the inability to decipher cause and effect at this timescale.
A need for coordinated multi-model simulations, data analyses and diagnostics to investigate the Arctic Amplification impacts on the mid-latitudes circulation and climate was evident, and the Polar Amplification Model Intercomparison Project (PAMIP), co-designed by MPI-M scientists, was established (Smith et al., 2019). The first coordinated analysis of the PAMIP experiments performed with 16 state-of-art atmospheric general circulation models (including ECHAM6) has revealed a robust but weak impact of Arctic Amplification on midlatitude weather and climate (Smith et al., 2022). The MPI-M scientists found that the response to Arctic sea ice loss and consequent Arctic Amplification consists of a weakening of mid-latitude tropospheric westerly winds and an equatorward shift of the storm tracks, consistent with a negative phase of the North Atlantic Oscillation. This tropospheric response occurs in all models, albeit with a wide inter-model spread in its strength. The inter-model spread in westerly wind strength response is linked to the spread in midlatitude eddy momentum feedback, a feedback 1.2 to 3 times too weak in the models (Fig. 4). Consequently, the simulated response likely underestimates the atmospheric circulation response to Arctic-sea ice loss and consequent Arctic Amplification. This raises the question how well critical processes, such as atmospheric blocking, that influence the response to Arctic Amplification are represented in climate models.
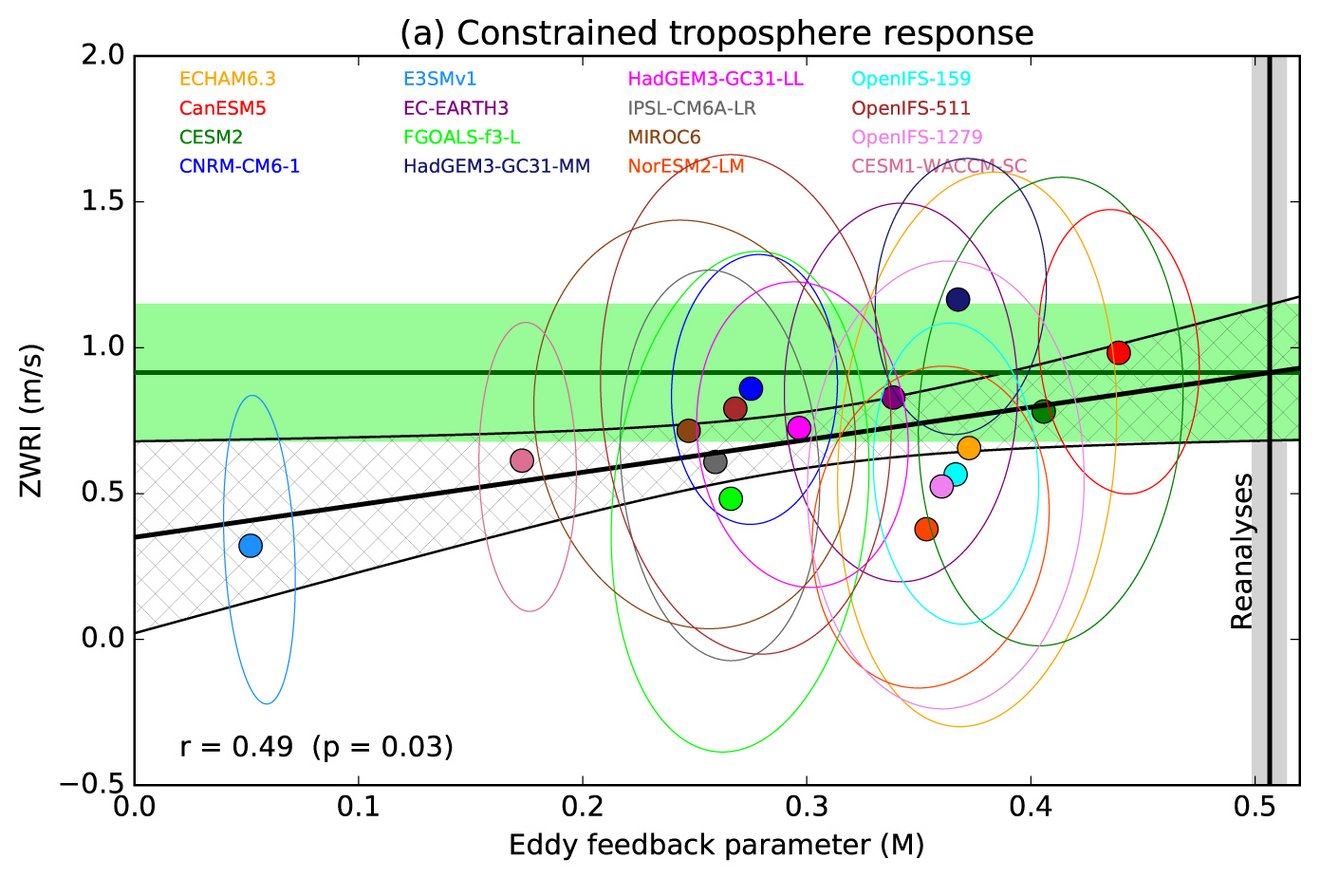
Despite the crucial contribution of blocking in understanding the dynamics causing climate extremes over the high latitudes and possible links to circulation anomalies in lower latitudes, the investigation is impeded by uncertainties related to definitions of blocking itself. A universally accepted definition for blocking and a single theory that explains its dynamics of formation, maintenance, and decay, are still lacking. Therefore, a plethora of blocking indices has been developed; each recognizes different aspects of the phenomenon, and different indices often yield diverging climatologies of blocking action. Such discrepancies are particularly noteworthy in the case of high-latitude blocking (HLB) activity and can have a significant impact in the study of Arctic blocking anticyclones that are important drivers of the summertime Arctic sea-ice melting. For example, the role of Greenland blocking in driving summertime melting of Greenland ice has been found to be of paramount importance.
The disagreement in HLB detection is exemplified when the blocking climatologies obtained by two prominent techniques are compared: the potential vorticity – potential temperature (PV-θ) blocking index (Pelly and Hoskins, 2003) and the absolute geopotential height (AGH) reversal method (Davini et al., 2012). The latter algorithm yields strikingly lower winter HLB activity over Greenland and the Bering Straits in comparison to the former (Fig. 5). The discrepancy is resolved after modifying the blocking detection criteria employed by the AGH method for latitudes higher than 60°N, resulting in the convergence of climatology, interannual variability, and trends of HLB between the two methods (Tyrlis et al., 2021). The better tuned AGH method can be valuable tool for the estimation of reliable HLB trends in climate model projections and crucial for the better understanding of the Arctic Amplification and mid-latitude climate links.
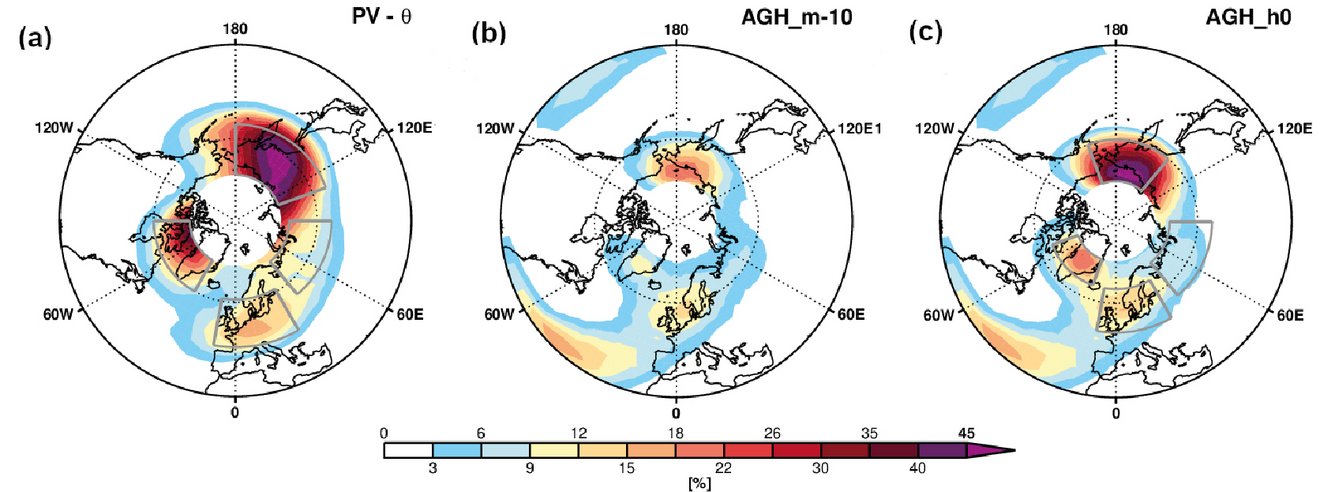
To summarize, on the shorter timescale (daily-to-subseasonal) the midlatitude atmospheric dynamics substantially impacts the Arctic weather and climate. On the longer time scale, the first coordinated multi-model study demonstrates a robust but relative weak influence of the Arctic on the midlatitude weather and climate. Whether changes in the Arctic may contribute substantially to changes in midlatitude variability in the future, especially when other influences – such as warming in the tropics – may play a role, is still unknown. The current state-of-the-art models underestimate the Arctic-Midlatitude linkages, perhaps a manifestation of the models underestimating the predictable part of climate variability over the Atlantic sector (Scaife and Smith, 2018). Furthermore, the non-stationarity caused by global warming raises the question of how the teleconnections and impacts between the Arctic and the mid-latitudes might change in the future.
References
A tug-of-war over the mid-latitudes. Nature Communications 10, 5578 (2019). doi:10.1038/s41467-019-13714-0
Bader, J., Mesquita, M., Hodges, K., Keenlyside, N., Osterhus, S., and Miles, M. (2011) A review on Northern Hemisphere sea-ice, storminess and the North Atlantic Oscillation: Observations and projected changes. Atmospheric Research, 101, 809-834. doi:10.1016/j.atmosres.2011.04.007.
Davini, P., Cagnazzo, C., Gualdi, S., and Navarra, A. (2012) Bidimensional diagnostics, variability, and trends of Northern Hemisphere blocking. Journal of Climate, 25, 6496–6509, 2012. doi:10.1175/JCLI-D-12-00032.1
Doblas-Reyes, F. J,. A. A. Sörensson, M. Almazroui, A. Dosio, W. J. Gutowski, R. Haarsma, R. Hamdi, B.Hewitson, W-T. Kwon, B. L. Lamptey, D. Maraun, T. S. Stephenson, I. Takayabu, L. Terray, A. Turner, Z. Zuo (in press) Linking Global to Regional Climate Change. In: Climate Change 2021: The Physical Science Basis. Contribution of Working Group I to the Sixth Assessment Report of the Intergovernmental Panel on Climate Change [Masson-Delmotte, V., P. Zhai, A. Pirani, S. L. Connors, C. Péan, S. Berger, N. Caud, Y. Chen, L. Goldfarb, M. I. Gomis, M. Huang, K. Leitzell, E. Lonnoy, J.B.R. Matthews, T. K. Maycock, T. Waterfield, O. Yelekçi, R. Yu and B. Zhou (eds.)]. Cambridge University Press. In Press.
Pelly J. L. and B. J. Hoskins (2003) A new perspective on blocking. Journal of the Atmospheric Sciences, 60, 743-755. doi:10.1175/1520-0469(2003)060%3C0743:ANPOB%3E2.0.CO;2
Scaife, A.A., Smith, D. (2018) A signal-to-noise paradox in climate science. npj Climate and Atmospheric Science 1, 28. doi:10.1038/s41612-018-0038-4
Smith, D. M., Screen, J. A., Deser, C., Cohen, J., Fyfe, J. C., García-Serrano, J., Jung, T., Kattsov, V., Matei, D., Msadek, R., Peings, Y., Sigmond, M., Ukita, J., Yoon, J. H., and Zhang, X. (2019) The Polar Amplification Model Intercomparison Project (PAMIP) contribution to CMIP6: Investigating the causes and consequences of polar amplification. Geoscientific Model Development, 12, 1139-1164. doi:10.5194/gmd-12-1139-2019
Smith, D.M., R. Eade, M. B. Andrews, H. Ayres, A. Clark, S. Chripko, C. Deser, N. J. Dunstone, J. Garcia-Serrano, G. Gastineau, L. S. Graff, S. C. Hardiman, B. He, L. Hermanson, T. Jung, J. Knight, X. Levine, G. Magnusdottir, E. Manzini, D. Matei, M. Mori, R. Msadek, P. Ortega, Y. Peings, A. A. Scaife, J. A. Screen, M. Seabrook, T. Semmler, M. Sigmond, J. Streffing, L. Sun, A. Walsh (2022) Robust but weak winter atmospheric circulation response to future Arctic sea ice loss. Nature Communications 13, 727. doi:10.1038/s41467-022-28283-y.
Tyrlis, E., Manzini, E., Bader, J., Ukita, J., Nakamura, H., and Matei, D. (2019) Ural Blocking driving extreme Arctic sea ice loss, cold Eurasia and stratospheric vortex weakening in autumn and early winter 2016-2017. Journal of Geophysical Research Atmosphere, 124, 11313– 11329. doi:10.1029/2019JD031085.
Tyrlis, E., Bader, J., Manzini, E., Ukita, J., Nakamura, H., and Matei, D. (2020) On the role of Ural Blocking in driving the Warm Arctic – Cold Siberia pattern. Quarterly Journal of the Royal Meteorological Society, 146, 2138-2153. doi:10.1002/qj.3784.
Tyrlis, E., Bader, J., Manzini, E., and Matei, D. (2021) Reconciling different methods of high-latitude blocking detection. Quarterly Journal of the Royal Meteorological Society, 147, 1070-1096. doi:10.1002/qj.3960.
Contact
Dr. Daniela Matei
Max Planck Institute for Meteorology
Email: daniela.matei@ mpimet.mpg.de
Dr. Elisa Manzini
Max Planck Institute for Meteorology
Email: elisa.manzini@ mpimet.mpg.de
Dr. Jürgen Bader
Max Planck Institute for Meteorology
Email: juergen.bader@ mpimet.mpg.de
Prof. Dr. Evangelos Tyrlis
Jetzt am: National and Kapodistrian University of Athens
Email: etyrlis@ phys.uoa.gr